Advanced Nuclear 101
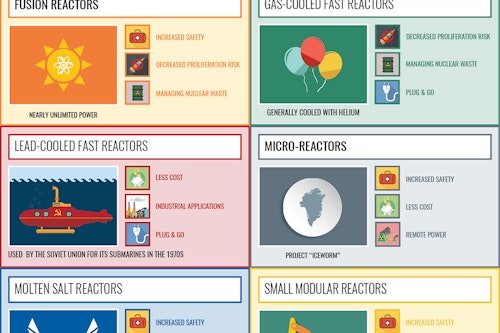
Even the phrase “advanced nuclear” can be intimidating. But you don’t need a Ph.D. to understand the basic differences between the various advanced reactors under development in this burgeoning part of the clean energy sector. In this primer, we offer some background on the advanced reactor space and explain the differences between the nine different types of advanced reactors currently under development. We provide the basic information that policymakers need to understand the various coolants, fuels, reaction types, and sizes that could provide many paths to a single destination: clean, affordable, and reliable electricity.
What is Advanced Nuclear?
If you are following emerging clean energy technologies in the United States or the energy and climate hearings on Capitol Hill, terms like “molten salt reactor” get thrown around a lot. And that’s just the beginning—how much do you know about “pebble bed reactors” or “lead-cooled fast reactors”? Fear not. Third Way has put together this primer on the nine emerging reactor types, with background on where this new industry came from, why it’s important, what differentiates each new technology, and how to judge their relative benefits.
This industry is real, and it is growing. In our June 2015 report, “Introducing the Advanced Nuclear Industry,” we identified nearly 50 advanced reactor projects backed by well over $1 billion in private capital.1 The industry is, however, in urgent need of help from Washington. If it gets it, we could see a set of breakthrough technologies that can power the world and address the climate crisis. But that won’t happen unless policymakers understand something about this technology and the promise that it holds.
The Birth of an Industry
When looking out on the dry desert plain of Idaho or down a long valley in Tennessee, you might see something you don’t expect: a smattering of buildings and structures that housed advanced nuclear reactors cooled with liquid metals, molten salts, and high temperature gasses. These so-called Generation I reactors, long retired and decommissioned, serve as a reminder of the promise advanced nuclear reactors once held for producing zero-carbon electricity and the opportunity they offer to address the energy and climate challenges we are facing today.
Today’s development of a new generation of advanced reactors, often referred to as Generation IV, builds on the government-funded R&D that gave us those reactors (and others) in the 1950s and 60s. In the golden age of nuclear innovation, the U.S. established the National Reactor Testing Station in Idaho, a predecessor of Idaho National Laboratory, and built more than 50 nuclear reactors.2 Additionally, a number of reactors were built at other national laboratories and sites including Argonne, Oak Ridge, Los Alamos, Brookhaven, Hanford, and Savannah River. As we described in a Brookings Essay on advanced reactors, U.S. policymakers were “locked in what they thought of as a life-or-death race with the Soviet Union, and they aimed to be first in every aspect of scientific inquiry, especially those that involved atom splitting.”3
The age of nuclear innovation slowed to a halt, however, when the head of the U.S. Navy’s nuclear fleet, Admiral Hyman Rickover, decided that the Navy should exclusively use light water reactors (LWR) to power submarines and aircraft carriers. The commercial sector followed the Admiral’s lead. From the late 1960s through today, almost every nuclear power plant we built (and most built worldwide) uses light water (that is, normal water) pumped under high pressure to both keep the nuclear reactor cool and to transfer heat from the reactor to the steam turbines that generate electricity. Because of their high operating pressure and ever-increasing size, these reactors require enormous steel pressure vessels that can only be purchased from a tiny number of global producers, all outside the U.S. As a result of this and other complexities, constructing these large reactors is a slow and very costly process.4
Today, the U.S. relies on almost 100 LWRs to generate 20% of our electricity and 63.3% of our carbon-free electricity.5 These reactors, located individually or combined in a group of two or three as part of a larger power plant, generate between 500 and 1,400 megawatts (MW) of electricity each.6
But in an era of advanced materials, supercomputing, and modular construction, different options are emerging. A new generation of engineers is picking up the mantle of innovation from their 60s-era forebears, and, motivated by climate change, is developing advanced reactor designs that can provide affordable, clean, safe power. They are considering how to use coolants other than light water; how to operate at normal atmospheric pressure; how to use physics in addition to engineering to keep reactors safe; and how to make reactors small enough (from 3 to 250 MW) to be mass-produced in factories, significantly slashing construction costs and saving time.
How to Use This Paper
This paper provides policymakers a basic introduction to the different technologies under development and illustrates the need for further private and public sector research into next generation nuclear energy. For ease of use, we've linked to the definition of key words related to nuclear energy.
The reactors being designed by individual companies or institutions will differ, even if they use the same coolant or basic design. Because of these differences, many of which are proprietary and unavailable for public evaluation, this paper was not developed to assess the feasibility of any of these reactor types or predict the likelihood for commercialization.
What Types of Technologies Are There?
The Basic Differences
The 48 North American companies and entities that we identified are working on nine distinct types of advanced reactor designs. Today’s reactors, and eight of the nine advanced reactors detailed below, use fission reactions: splitting an isotope to release neutrons that create heat energy, which can be harnessed to generate electricity.7 This can be done in two basic ways:
- Thermal reactors use moderators like water to slow down neutrons, which allows fission to happen more easily.8 Most commercial reactors today are thermal and the vast majority of the 16,000 cumulative reactor-years of experience in operating commercial nuclear power comes from thermal reactors.9
- Fast breeder or neutron reactors, collectively known in this paper as fast reactors, keep the neutrons moving quickly, which makes the fission reaction more efficient and in some cases can actually breed more nuclear fuel. These reactors can consume the most dangerous waste of light water reactors thereby reducing the total quantity of waste requiring deep geologic disposal.10
Of the nine advanced nuclear technologies we review, the main difference between five of the reactor types in this primer is the coolant used to keep the reactor operating safely. Two others are distinguished by their size relative to modern, light water reactors. One is distinguished by temperature. And, finally, fusion would use a completely different physical reaction to produce power.
The Common Benefits
To succeed, the next generation of advanced reactors must do some things better or cheaper than their light water predecessors. Here are the benefits that most of them offer:
Increased Safety: Because many advanced reactor designs do not use high pressure, or even water as a coolant, they can rely on the passive physics of the reactor system (rather than active safety systems) to shut the reactor down and remove residual heat in the event of an accident or malfunction. An example is the “plug and drain” system. If a fluid-filled molten salt reactor gets too hot, it will melt a plug located at the bottom of the reactor, and the molten salt will drain down to a catch basin where it will cool on its own. This all happens because of gravity—no pumps, external power, or human intervention is required.
Decreased Proliferation Risk: Most advanced reactor designs would lower the risk of proliferation by consuming the plutonium they produce or simply not producing it in significant amounts. These reactors can also take the plutonium stockpiles from countries that have nuclear weapons programs and use that plutonium for power production instead. This would continue the momentum of the Megatons to Megawatts program that ended in 2013, where the U.S. purchased approximately 20,000 nuclear bombs worth of excess highly enriched uranium from Russia and used it as fuel for American civilian nuclear reactors.11 Other advanced designs can use depleted uranium, the waste remnants of the uranium enrichment process. This would eliminate the need for centrifuges—also required for the production of highly enriched, weapons-grade uranium—and would dramatically reduce the proliferation risk from countries like Iran that might use a civilian nuclear program as cover for military ambitions. In fact, allowing Iran to maintain these centrifuges was a central sticking point in the nuclear negotiations with the U.S.
Plug & Go: Most civilian reactors currently in operation around the globe need to be refueled every 18 to 24 months. This process requires significant infrastructure to ensure that the refueling is done safely and the spent fuel is secured against accident or theft. Reactors also go offline for about 40 days during the refueling process, costing their operators money and temporarily eliminating a reliable and major source of electricity. Some advanced reactors are being developed to be “plug go,” meaning that once the reactor is installed on site, it doesn’t need to be refueled for up to twenty years. This extended fuel cycle is a significant benefit to countries that don’t want or cannot afford to build the necessary infrastructure, such as enrichment, fuel fabrication, or nuclear waste facilities. It also protects against nuclear fuel being diverted for weapons development.
Managing Nuclear Waste: Spent fuel, a type of nuclear waste, is a challenge for today’s reactor operators and the federal government. While operators are locally storing spent fuel safely, the process is costly and the public remains concerned about the continued production of this type of nuclear waste that lasts for thousands of years. Many advanced reactor designs would address these concerns by actually consuming spent fuel, dramatically reducing the amount of waste requiring storage. Other advanced reactors, breeder reactors, would help manage nuclear waste by using fuel much more efficiently than current reactors and by actually creating new nuclear fuel. This could significantly reduce the real, but manageable environmental challenges caused by trying to store spent nuclear fuel for centuries.
Bridging Technology: It would be a significant leap to go from the large, light water reactors in use today to smaller, advanced reactors that use liquid metal or high temperature gas as a coolant. There are technologies under development that bridge the gap by using light water as a coolant and applying it to smaller, modular reactors. In the near term, this would be easier for regulators to evaluate and utilities to deploy and operate, while addressing some of the cost, scaling, and safety challenges of large light water reactors operating today.
Industrial Applications: Today, fossil fuels create the very high temperatures needed for industrial furnaces, which are used in sectors such as iron and steel, chemicals, and cement. This results in a portion of the approximately 1,400 megatons of direct greenhouse gas emissions annually in the U.S. alone.12 Renewables and existing nuclear reactors cannot efficiently produce the high temperatures needed to replace these furnaces. Some advanced reactors would safely operate at temperatures high enough to supplant fossil fuels in industrial processes and to produce electricity as well.
Scaling Size: Some reactors are classified as advanced simply because of the size. Today, most reactors are built to generate between 1000 and 1200 MW of electricity. The majority of markets in the developed world do not need additional electricity at that scale. Advanced nuclear developers are designing small modular reactors and micro-reactors, which are designed to generate between 10 and 200 MW per reactor. These small modular and micro-reactors could be built in a factory and then shipped to the construction site for a relatively quick installation. Because of their modular design, operators can scale the power plant to meet their changing needs, adding new reactors more quickly, cost effectively, and in smaller generation increments as demand grows. The modular design also has the potential to reduce the security demands on the operator, as the footprint of the site could be much smaller than today’s power plants. Many of the advanced reactor designs are also intended to be installed underground, making them smaller, harder targets for a terrorist or other attack.
Less Cost: Developers are working to create reactors with simpler designs, modular construction, scaling, and other innovations to be cost competitive with fossil fuels. A number of the benefits we list here also contribute to lower overall costs, including passive safety systems, increased time between refueling, and improved reliability.
Remote Power: Some micro-reactors are being designed specifically for the most remote locations—think mining operations, military installations, or isolated villages. These reactors are completely self-contained, generate relatively small amounts of electricity (around 2 to 5 MW), and can be easily brought to remote locations, installed, and left to operate for years at a time without intervention. Such a reactor could power a defense facility, avoiding the costly and often dangerous practice of transporting millions of gallons of liquid fuels over inhospitable terrain, or relying on a vulnerable public grid.
Unlimited Power: While existing reactors need to be refueled every 18 to 24 months and some advanced reactors can operate for as many as 20 years between refueling, there is the potential to develop a reactor that could run essentially in perpetuity. These designs would use innovative fuel cycles or simply the physics of the reactors to re-use the waste produced from the reaction process to operate for as long as a century without having to go offline for a sustained period of time. A handful of developers are working on fusion, rather than fission, reactors. While much more complicated and still far off from commercialization, fusion reactors, which use hydrogen as fuel, could have fuel that is nearly unlimited and inexpensive to produce, without the problem of spent fuel waste to manage, recycle, or secure.13
What Will Happen Next?
The Race for the Next Generation of Nuclear
The dozens of companies and research centers working on advanced reactors must resolve real materials, design, and financing challenges before they are ready to build test reactors, let alone commercialize their technology. It is too early in the process, and there are too many technical and financial hurdles to overcome for us to predict exactly when or which technology might find commercial success. But we do know that the global demand for cleaner, affordable, and reliable energy is spurring innovation in nuclear energy unseen since the 1960s. With the right combination of research, private funding, and federal policies, advanced nuclear technology has the potential to play a major role in addressing climate change and growing energy demand in the 21st century.
The Federal Government's Role
The federal government must partner with the private sector to help develop this potentially world-changing technology. This includes providing access to federal facilities to demonstrate new designs and a straightforward pathway for licensing approval to operate advanced reactors.
Developers are moving from “paper reactors”—that is, designs on computer hard drives—to testing materials and building prototypes or demonstration reactors. To do this, they need access to test reactors technical expertise, and sites to safely construct and operate non-commercial versions of their reactors. The Department of Energy and U.S. national labs have the facilities, expertise, and at several locations, the secure land, to help developers move their designs forward. In fact, more than 50 reactor designs were built and tested at U.S. federal facilities in the 1960s. The model for private-public partnerships, however, should be streamlined. We need to make it easier for small businesses to work with the government on nuclear innovation, clarify regulations, and provide some federal funding for the facilities.
At the same time, the Nuclear Regulatory Commission (NRC) should modernize the pathway for licensing approval for companies seeking to commercialize advanced reactors. Start-ups and even large companies with first-of-a-kind reactors cannot raise the hundreds of millions of dollars in private capital needed today to pay for licensing or engage in a decade or longer review process. The NRC should not have to begin planning how to evaluate dozens of different designs of paper reactors, many of which will never get to the licensing process. The NRC recognizes these challenges and convened a conference in September 2015 with DOE to consider options for regulating advanced technologies that provide a reasonable path to licensing while meeting its mission to ensure the safety of civilian nuclear operations in the U.S.14 Time, however, is of the essence. The longer the U.S. goes without a timely, predictable, affordable, and safe licensing path for the companies that emerge and are ready to commercialize their advanced reactors, the more likely it is that another country will be the home to this technology.
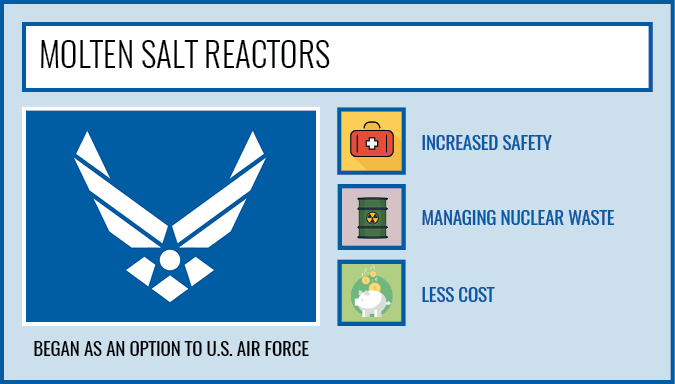
Developers: Flibe Energy, GEMSTAR, MIT, Oak Ridge National Lab Terrestrial Energy, Thorcon, Thorenco, Transatomic Power, UC-Berkeley
Highlighted Benefits: Increased Safety, Managing Nuclear Waste, Less Cost
Molten salt might not sound like the ideal substance to keep a nuclear reactor cool. But don’t think really hot table salt. Instead, the primary coolant in the core of a molten salt reactor (MSR) is a fluoride salt heated by the nuclear reaction to a liquid state at around 650 degrees Celsius. The ability of the molten salt to remain stable at high temperatures helps the reactors get more energy per ounce of fuel than our current reactors. The uranium or thorium fuel for an MSR—which can be a fast or thermal reactor, depending on design—can either be placed in a solid rod, just as it is in reactors operating today, or it can be dissolved directly into the molten salt itself to flow through the core of the reactor where the fission takes place. A key challenge is the corrosive nature of molten salt. Modern reactor designers will need to test new materials to determine if they can safely withstand the salt, as well as the radiation and high temperatures that every nuclear reactor generates.
The idea of using molten salt as a coolant in a nuclear reactor is not new. In the wake of the Manhattan Project, each branch of the U.S. military researched how to harness the atom for more peaceful purposes. The U.S. Air Force first developed the Molten Salt Reactor in the 1950s.15 While that reactor was never used in flight, research continued under non-defense projects such as the Molten Salt Reactor Experiment (MSRE) at Oak Ridge National Lab in the 1960s.16 Oak Ridge scientists actually built and operated an MSR for 20,000 hours, though they never connected it to a turbine to make electricity. This experiment yielded four years of extremely valuable data, proving the physics of how these reactors operate. Modern developers are now using these data to build a 21st century version of this technology.
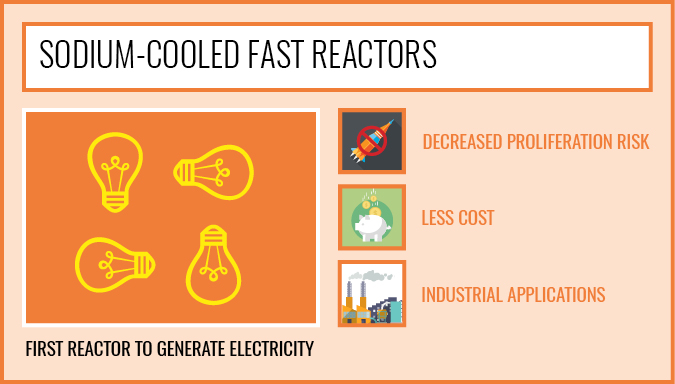
Developers: Advanced Reactor Concepts, Argonne National Laboratory, GE-Hitachi, TerraPower
Benefits: Decreased Proliferation Risk, Managing Nuclear Waste, Less Cost, Industrial Applications
Sodium-cooled fast reactors (SFR) use a liquid metal as a coolant, similar to how the molten salt reactor (MSR) uses molten salt to keep the nuclear reactor core at a constant temperature. The metal in these reactors is not a red-hot, glowing piece of iron; it is actually liquid sodium. SFRs use only sodium as a coolant, rather than the fluoride salts used by MSRs and, also unlike MSRs, the fuel is placed in rods and cannot be dissolved into the sodium coolant. SFRs are fast reactors because the neutrons that they use to create a nuclear reaction have more energy than the neutrons used in today’s light water reactors. The design also enables SFRs to use the uranium and plutonium from spent fuel as its fuel. Today, Russia operates an SFR known as BN-600 and it is constructing BN-800, which had its first measurable and controllable reaction on June 27, 2014; both reactors use liquid sodium as a coolant.17 Bill Gates has invested in a company, TerraPower, which is developing an SFR18 and is currently conducting a wide range of tests in the U.S., Korea, and even Russia, which has some testing facilities that are not available in the U.S.19
The world’s first electricity-generating nuclear power plant was an SFR. Electricity generated by the SFR known as Experimental Breeder Reactor No. 1 (EBR-I), located at the Idaho site, powered four light bulbs in 1951.20 EBR-I operated for nearly 15 years, providing extensive experimental data to the nuclear research community.21 Sodium reactors were not commercialized, in part, because while sodium excels at keeping a reactor cool, it has some unique challenges in everyday operating situations: it reacts badly with water (it explodes) and air (it burns). Fortunately, the United States has been operating research SFRs for decades. This experience has taught us more about how to manage chemical reactions between sodium and water or air.22

Developers: Argonne National Laboratory, Gen4 Energy, LakeChime, Westinghouse
Highlighted Benefits: Increased Safety, Managing Nuclear Waste, Less Cost, Industrial Applications, Plug & Go
Most people are familiar with lead’s interaction with radiation, even if they don’t know it. That’s because most of us have worn a lead-lined vest to protect vital organs when getting an x-ray. Lead stops or reflects radiation, such as x-rays or neutrons, from traveling where we don’t want it to go. In the case of a lead-cooled fast reactor (LFR), liquid lead flows through the reactor and reflects neutrons away from the outside of the reactor and back into the core. LFRs are also able to use the uranium and plutonium from spent fuel as its fuel. Many of these reactors are small and modular with natural convection using the reactors’ own heat to move the coolant through the reactor, rather than requiring pumps to circulate the coolant in the case of an emergency as light water reactors do. Developers believe that, by using lead as a coolant, an LFR could be built in factories, shipped to the operating location, and buried underground to operate for as long as 20 years without needing to be shut down for refueling.
LFRs date back to the 1950s and were most widely used deep below the ocean.23 In the 1970s, the Soviet Union used LFRs for its nuclear submarines because the reactors were comparatively light.24 Lawrence Livermore National Laboratory recently researched LFRs because these reactors could generate electricity without producing spent fuel that could be turned into weapons-grade materials that posed a proliferation risk. One reason LFRs have not been commercialized is that it is hard to monitor the state of a reactor core surrounded by lead. Today, companies researching this technology are working on ways to “see” through the lead for maintenance of the reactor and the natural convection patterns of lead in a reactor.
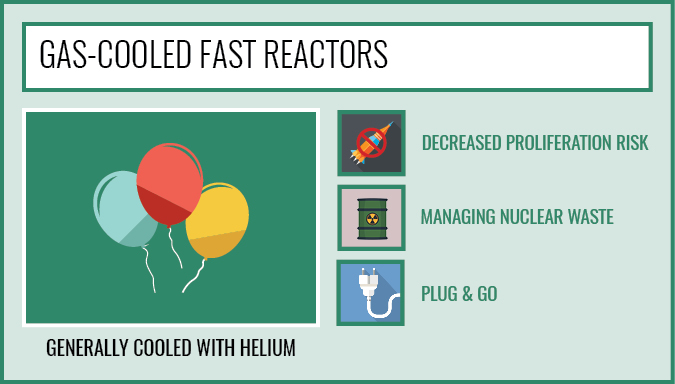
Developers: General Atomics, Hybrid Power Technologies
Highlighted Benefits: Decreased Proliferation Risk, Managing Nuclear Waste, Less Cost, Plug & Go
Gas-cooled fast reactors (GFR) are generally cooled with helium. Unlike in balloons, the helium circulating through the reactor is between 650 and 850 degrees Celsius. This process enables a GFR to use fast neutrons to power the fission reaction, releasing more of the energy in the fuel than many other advanced reactors and today’s light water reactors. The design also enables GFRs to use the uranium and plutonium from spent fuel as its fuel. As with other advanced reactor designs, GFR developers are seeking to ensure that the materials used to construct the reactor can hold up over long periods of time to the extremely hot gasses moving inside. There are advanced materials that could be the answer to this challenge, but more testing is required. GFRs are also safer than today’s LWRs because they rely on passive safety features that use physics, rather than active safety systems that are more susceptible to human error or failure.
The U.S. first began operating gas-cooled reactors in 1967.25 The two units, which were not fast reactors, were used to help find new ways to make reactor operations more efficient and use newly developed materials to improve reactor operation. The reactors remained in operation until 1989.
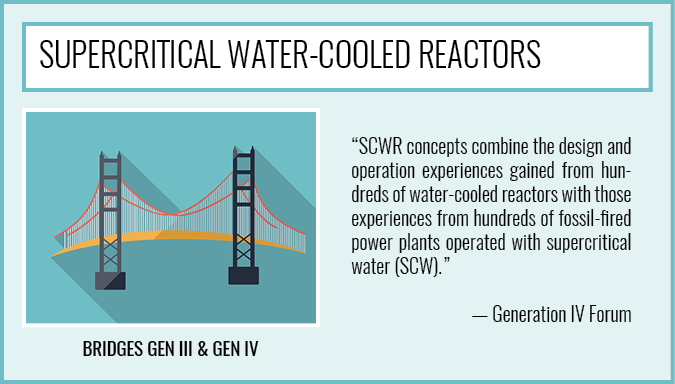
Developers: Atomic Energy of Canada Limited (AECL)
Highlighted Benefits: Managing Nuclear Waste, Bridging Technology
Supercritical water-cooled reactors (SCWR) are similar to today’s light water reactors but operate at a higher temperature and at a higher pressure, enabling them to generate electricity at a higher efficiency. This process is known as “supercritical,” where water is above the critical temperature and pressure point where liquids and gases are distinctly different. This makes the transfer of heat—and therefore the operation of the turbines generating electricity—more efficient. This concept would combine the decades of experience from supercritical coal plants with the decades of experience from operating light water reactors.26 An SCWR could be a fast reactor or a thermal reactor.
Experiments to develop an SCWR first began in the 1950s and 1960s. The design is viewed by some as bridging the gap between today’s light water reactors, known as Generation III+ reactors, and the next generation of technologies that use coolants other than water (many of which we discuss in this paper). The challenge, however, is that the costs to build an SCWR are likely to be higher than today’s reactors because of the additional materials needed to manage operations and higher temperatures and pressures. Additionally, SCWRs include many of the problems of LWRs, such as inefficient fuel use and safety concerns. During the early 2000s, the Generation IV Forum, a collaboration of 13 countries interested in developing advanced nuclear reactors, developed reference designs for an SCWR, but little commercial interest has emerged for this concept.27
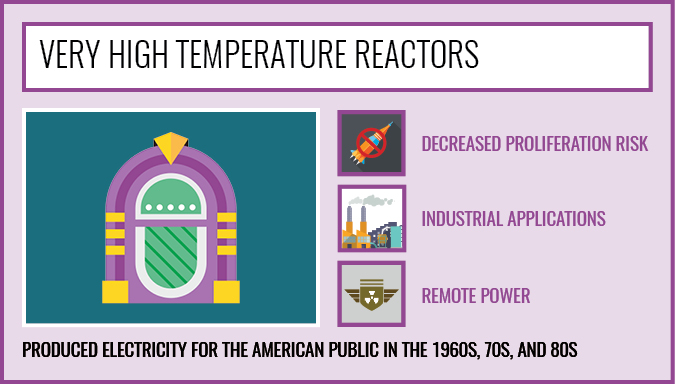
Developers: Areva, General Atomics, MIT, Next Generation Nuclear Plant, Northern Nuclear, X-Energy, Starcore Nuclear
Highlighted Benefits: Increased Safety, Decreased Proliferation Risk, Industrial Applications, Remote Power
The Very High Temperature Reactor (VHTR), also called a High Temperature Gas Reactor (HTGR), is a concept designed around a specific purpose—creating high temperatures for industrial heat processes—rather than a technical coolant difference. As noted above, the vast majority of industrial processes needing heat rely on fossil fuels, which contribute to U.S. greenhouse gas emissions. This cannot be replaced by renewables, which do not produce sufficient heat for industrial processes.
The VHTR, a thermal reactor, uses graphite (yes, just like pencils) as a moderator to slow the neutrons down. (This distinguishes the VHTR from most other advanced nuclear designs, which are fast reactors, such as GFRs.) Typically using low enriched uranium fuel, sometimes in the form of “pebbles” or in a hexagon shaped block called a “prismatic block”, the reactor heats the helium gas coolant to temperatures typically between 800 and 1,000 degrees Celsius, higher than temperatures used by GFRs. This heat is then used by industrial furnaces to produce hydrogen, desalinate water, or refine petrochemicals.28
In 1947, Oak Ridge National Laboratory began considering the need for a high temperature gas reactor. This helped lead to the development of the HTGRs that generated electricity at Fort St. Vrain in Colorado, which operated from 1979-1989,29 and Peach Bottom in Pennsylvania, which operated from 1967-1974.30 Both the Chinese and U.S. governments remain interested in HTGR technology, with China developing a pebble-bed version of the reactor. In 2007, the U.S. government launched the Next Generation Nuclear Program (NGNP), in conjunction with an industry alliance of partners, to commercialize an HTGR.31 NGNP, however, has faced management and leadership challenges that have delayed funding for a demonstration reactor.

Developers: Holtec, mPower, NuScale, RADIX, Westinghouse
Highlighted Benefits: Increased Safety, Less Cost, Industrial Applications, Bridging Technology, Scaling Size and Cost
Not all advanced reactor developers are relying on exotic coolants or fast neutrons to compete in the commercial market. Some are using tried and true light water technology but putting it into reactors that are far smaller and simpler than those operated by utilities today. These small reactors would be built as modular units in factories, not custom built on-site at the power plant, significantly reducing the cost of manufacturing and construction. These small modular reactors (SMR) would generate less than 300 MW,32 compared with the typical reactor operating in the U.S. today that have an average capacity of 1,000 MW, and could be either thermal or fast.33 SMRs would also be safer than today’s LWRs by utilizing passive physics to shut down the reactor, rather than active engineered systems. There is a need for SMRs from utilities in the U.S. and abroad that could not afford or do not have the electricity demand to justify a large, modern light water reactor. NuScale recently published costs for their SMR design in the range of $1 to $3 billion, compared to $14 to $16 billion for current large plants.34
The history of SMRs spans back to one of the original goals of nuclear innovation in the U.S.: the use of nuclear power to propel and power submarines. But since then, the size of civilian reactors has only gone one direction: up. In fact, according to the World Nuclear Association, reactors have grown from around 60 MW when the first civilian nuclear reactors came online in the 1950s to more than 1,600 MW for reactors coming online today, because this enables reactor manufacturers to take advantage of economies of scale in construction and operation.35 The challenge is that even with economies of scale, only a handful of utilities in the U.S. or even nationally owned utilities around the world can afford to finance the massive cost of constructing a 1,000 or more MW reactor.36

Developers: CityLabs, Dunedin, Gen4 Energy, LakeChime, Toshiba, UPower, Widetronix
Highlighted Benefits: Increased Safety, Less Cost, Remote Power
Today, military bases, mining facilities, and villages in remote locations rely on carbon-emitting fossil fuels, often diesel or fuel oil, to be transported to them at extremely high cost (as much as 30 cents per kilowatt hour) to provide all of their energy needs.37 For military bases, this supply chain is a very real risk to operations. Micro-reactors, generating as little as 2 MW of electricity, sufficient to power up to 2,000 homes, or as much as 50 MW, are being designed with an eye toward meeting these locations’ power needs at a lower operating cost than fossil generation.38 Most micro-reactors use water as a coolant, though some of the advanced reactors with unique coolants can also be scaled down to a micro size; micro-reactors can also be either thermal or fast. The advantage these reactors could offer is that they, like SMRs, would be manufactured at a factory and shipped, with fuel, to remote locations where they could operate for sustained periods of time without the need for refueling. And instead of being refueled onsite, the entire reactor would be removed and replaced with a new unit.
The U.S. military has been one of the most vocal proponents of micro-reactors to power small and remote installations. In fact, from 1959 to 1966, the Army operated a two MW reactor to power a semi-secret military installation in northern Greenland known as Project Iceworm.39 More recently, the town of Galena, Alaska, which is 270 miles north of Fairbanks, considered installing a small reactor to provide electricity to its 600 residents.40 The project in Galena stalled due to cost and regulatory issues, but the state of Alaska and many individual communities in the state, along with military bases, mines, and other remote communities, continue to look at the technology as a way to provide cost-effective, zero-emissions power.41

Developers: General Atomics, General Fusion, Helion, HyperV Technologies, ITER, Lockheed-Martin, NIF, NumerX, Tri Alpha
Highlighted Benefits: Unlimited Power, Increased Safety, Managing Nuclear Waste
All of the nuclear reactors in operation around the world, as well as the majority of advanced reactor technologies under development, rely on fission—breaking atoms apart—to produce heat and generate electricity. This is a well-understood process that has been in use for more than half a century. A handful of companies are pursuing the so-far elusive goal of producing heat and generating electricity by fusing two atoms together, a process not surprisingly known as fusion. Fusion uses hydrogen (found in water) as fuel and is the process that powers our Sun and all stars in the universe. Described by the New Yorker as a “star in a bottle,” fusion produces temperatures so high (from 150 million to billions of degrees Celsius) no known material can contain it.42 This fundamental obstacle has prevented researchers from achieving fusion in a lab, let alone a commercial reactor. But the potential for unlimited energy that produces almost no radioactive waste is too great to ignore.
There are two main approaches for trying to accomplish this feat that literally powers the sun. One is to use very high-powered magnets to confine a superheated mixture called a plasma where the atoms fuse and produce energy. The second way is by using an intense set of lasers fired at a target of atoms, compressing them to the point of fusing, called inertial confinement.43 Developers are now looking at some hybrid options between these two approaches as well.
Fusion research technically began in the 1920s.44 By the 1950s, the needs of the Cold War shifted the research focus away from energy and towards weapon implications.45 Modern research, driven by the need to produce zero-carbon electricity and concerns about radiation, proliferation, and nuclear waste, has returned to trying to harness fusion for electricity generation, though the timeframe for achieving such a scientific breakthrough remains unknown.
In 2007, ITER was organized to build a large fusion plasma facility, called a tokamak, in France.46 ITER is supported by the U.S., China, the EU, India, Japan, Korea, and Russia. This multi-decade, multi-billion dollar project is expected to have first plasma in the late 2020s.
Endnotes
Sam Britton, “The Advanced Nuclear Industry,” Report, Third Way, June 15, 2015. Accessed October 29, 2015. Available At: http://www.thirdway.org/report/the-advanced-nuclear-industry.
United States, Department of Energy, “Idaho National Laboratory.” Accessed November 13, 2015. Available at http://energy.gov/em/idaho-national-laboratory.
Josh Freed, “Back to the Future: Advanced Nuclear Energy and the Battle Against Climate Change,” Essay, The Brookings Essay, Brookings, December 12, 2014. Accessed October 29, 2015. Available at: http://www.brookings.edu/research/essays/2014/backtothefuture#.
“Heavy Manufacturing of Power Plants,” World Nuclear Association. Accessed on October 29, 2015. Avaiable at: http://www.world-nuclear.org/info/Nuclear-Fuel-Cycle/Power-Reactors/Heavy-Manufacturing-of-Power-Plants/.
Keith Kohl, “Nuclear Energy Makes Huge Strides in the U.S. Energy Portfolio,” Energy and Capital, July 8, 2015. Accessed October 29, 2015. Available at: http://www.energyandcapital.com/articles/nuclear-energy-makes-huge-strides-in-the-us-energy-portfolio/4927; See also United States, Energy Information Administration, “Frequently Asked Questions: What is U.S. electricity generation by energy source?,” Accessed October 29, 2015. Available at: http://www.eia.gov/tools/faqs/faq.cfm?id=427&t=3.
United States, Energy Information Administration, “Frequently Asked Questions: How much electricity does a nuclear power plant generate?.” Accessed October 29, 2015. Available at: http://www.eia.gov/tools/faqs/faq.cfm?id=104&t=21.
United States, Department of Energy, “The History of Nuclear Energy.” Accessed October 29, 2015. Available at: http://energy.gov/ne/downloads/history-nuclear-energy.
Nick Touran, “What is a fast reactor?,” What is Nuclear?, September 2006. Accessed November 6, 2015. Available at: http://www.whatisnuclear.com/articles/fast_reactor.html.
“Safety of Nuclear Power Reactors,” World Nuclear Association. Accessed on November 17, 2015. Available at: http://www.world-nuclear.org/info/safety-and-security/safety-of-plants/safety-of-nuclear-power-reactors/.
Nick Touran, “What is a fast reactor?,” What is Nuclear?, September 2006. Accessed October 29, 2015. Available at: http://www.whatisnuclear.com/articles/fast_reactor.html.
“Megatons to Megawatts: Russian Warheads Fuel U.S. Power Plants,” NPR, December 11, 2013. Accessed October 30, 2015. Available at: http://www.npr.org/2013/12/11/250007526/megatons-to-megawatts-russian-warheads-fuel-u-s-power-plants.
United States, Environmental Protection Agency, “Sources of Greenhouse Gas Emissions: Industry Sector Emissions.” Accessed October 30, 2015. Available at: http://www3.epa.gov/climatechange/ghgemissions/sources/industry.html.
Dino Grandoni, “Start-Ups Take On Challenege of Nuclear Fusion,” New York Times, October 25, 2015. Accessed November 12, 2015. Available at: http://www.nytimes.com/2015/10/26/technology/start-ups-take-on-challenge-of-nuclear-fusion.html?_r=0.
United States, Nuclear Regulatory Commission, “NRC-DOE Advanced Non-Light Water Reactors Workshop,” Accessed October 30, 2015. Available at: http://www.nrc.gov/public-involve/conference-symposia/adv-rx-non-lwr-ws.html.
Neil Endicott, “Thorium-Fuelled Molten Salt Reactors,” Report, The Weinberg Foundation, June 2013, Sec. 1, p.3. Accessed October 30, 2015. Available at: http://www.the-weinberg-foundation.org/wp-content/uploads/2013/06/Thorium-Fuelled-Molten-Salt-Reactors-Weinberg-Foundation.pdf.
“Molten Salt Reactors.” World Nuclear Association. Accessed October 30, 2015. Available at: http://www.world-nuclear.org/info/Current-and-Future-Generation/Molten-Salt-Reactors/.
“Fast Neutron Reactors.” World Nuclear Association. Accessed on October 30, 2015. Available at: http://www.world-nuclear.org/info/Current-and-Future-Generation/Fast-Neutron-Reactors/.
TerraPower. Accessed on October 30, 2015. Available at: http://terrapower.com/.
“TerraPower: A Nuclear Energy Technology Company.” Accessed October 30, 2015. Available at: http://www.uxc.com/smr/Library%5CDesign%20Specific/TWR/Other%20Documents/TerraPower%20-%20A%20Nuclear%20Energy%20Technology%20Company.pdf.
United States, Argonne National Laboratory, “Argonne History – 1950s,” Photo. Accessed November 12, 2015. Available at: http://www.anl.gov/photos/argonne-history-1950s.
United States, Idaho National Laboratory, “An Energy Landmark: Idaho’s pioneering Experimental Breeder Reactor.” Accessed on October 30, 2015. Available at: http://www4vip.inl.gov/ebr/.
United States, Argonne National Laboratory, Catherine Westfall, “Vision and reality: The EBR-II story,” Article, Nuclear News, February 2004. Accessed November 12, 2015. Available at: http://www.ne.anl.gov/About/reactors/EBR2-NN-2004-2-2.pdf.
M. Tarantino, L. Cinotti, D. Rozzia, “Lead-Cooled Fast Reactor (LFR) Development Gaps,” Report, International Atomic Energy Agency. Accessed October 30, 2015. Available at: https://www.iaea.org/NuclearPower/Downloadable/Meetings/2012/2012-02-29-03-02-TM-FR/11a_Tarantino-Cinotti.pdf.
A.V. Zrodnikov, V.I. Chitaykin, B.F. Gromov, et al., “Use of Russian Technology of Ship Reactors with Lead-Bismuth Coolant in Nuclear Power,” International Atomic Energy Agency. Accessed October 30, 2015. Available at: http://www.iaea.org/inis/collection/NCLCollectionStore/_Public/31/058/31058476.pdf; See also “Nuclear-Powered Ships.” World Nuclear Association. Accessed October 30, 2015. Available at: http://www.world-nuclear.org/info/Non-Power-Nuclear-Applications/Transport/Nuclear-Powered-Ships/.
Andrew C. Kadak, “High Temperature Gas Reactors,” Briefing to Digital Power Capital, Massachusetts Institute of Technology. Accessed October 30, 2015. Available at: http://web.mit.edu/pebble-bed/Presentation/HTGR.pdf.
“Supercritical-Water-Cooled Reactor (SCWAR),” Generation IV International Forum. Accessed October 30, 2015. Available at: https://www.gen-4.org/gif/jcms/c_9360/scwr.
Generation IV International Forum. Accessed October 30, 2015. Available at: https://www.gen-4.org/gif/jcms/c_9260/Public.
“Nuclear Processed Heat for Industry,” World Nuclear Association. Accessed October 30, 2015. Available at: http://www.world-nuclear.org/info/Non-Power-Nuclear-Applications/Industry/Nuclear-Process-Heat-for-Industry/.
“Fort St. Vrain,” International Atomic Energy Association. Accessed October 30, 2015. Available at: https://www.iaea.org/PRIS/CountryStatistics/ReactorDetails.aspx?current=623.
“Peach Bottom Atomic Power Station,“ Exelon. Accessed October 30, 2015. Available at: http://www.exeloncorp.com/PowerPlants/peachbottom/Pages/profile.aspx.
United States, Department of Energy, “Next Generation Nuclear Plant,” Report to Congress, Next Generation Nuclear Plant, April 2010. Accessed October 30, 2015. Available at: http://energy.gov/sites/prod/files/4.4_NGNP_ReporttoCongress_2010.pdf.
“Small and Medium Sized Reactors (SMRs) Development, Assessment and Deployment,” International Atomic Energy Agency. Accessed October 30, 2015. Available at: https://www.iaea.org/NuclearPower/SMR/.
United States, Energy Information Agency, “Table 8.1: Nuclear Energy Overview,” Table. Accessed November 12, 2015. Available at: http://www.eia.gov/totalenergy/data/monthly/pdf/sec8_3.pdf.
“Construction Costs for a NuScale Nuclear Power Plant,” NuScale. Accessed November 12, 2015. Available at: http://www.nuscalepower.com/smr-benefits/economical/construction-cost.
“Small Nuclear Power Reactors,” World Nuclear Association. Accessed October 30, 2015. Available at: http://www.world-nuclear.org/info/Nuclear-Fuel-Cycle/Power-Reactors/Small-Nuclear-Power-Reactors/.
David Biello, “Is Spent Nuclear Fuel a Waste or a Resource?,” Scientific American, September 18, 2010. Accessed October 30, 2015. Available at: http://www.scientificamerican.com/article/is-spent-nuclear-fuel-waste-or-resource/.
Brian Wang, “UPower wants to make a container sized nuclear fission reactor with 2% of the development cost of small nuclear reactors and get regulatory approval by 2019,” Nextbigfuture, August 23, 2014. Accessed October 30, 2015. Available at: http://nextbigfuture.com/2014/08/upower-wants-to-make-container-sized.html.
Kyle Russell, “YC-Backed UPower Is Building Nuclear Batteries,” TechCrunch, August 18, 2014. Accessed October 30, 2015. Available at: http://techcrunch.com/2014/08/18/yc-backed-upower-is-building-nuclear-batteries/.
John Reed, “Inside the Army’s Secret Cold War Ice Base,” Defense Tech, April 6, 2012. Accessed October 30, 2015. Available at: http://defensetech.org/2012/04/06/inside-the-armys-secret-cold-war-ice-base/.
Jill Burke, “Galena’s nuclear option,” Alaska Dispatch News, March 14, 2010. Accessed October 30, 2015. Available at: http://www.adn.com/article/galena-s-nuclear-option.
Molly Rettig, “Why nuclear energy is on hold for Alaska,” News-Miner, January 23, 2011. Accessed October 30, 2015. Available at: http://www.newsminer.com/news/local_news/why-nuclear-energy-is-on-hold-for-alaska/article_51958987-2a69-5528-aa4b-fd2755913460.html.
Raffi Khatchadourian, “A Star in a Bottle,” The New Yorker, March 3, 2014. Accessed October 30, 2015. Available at: http://www.newyorker.com/magazine/2014/03/03/a-star-in-a-bottle.
“Nuclear Fusion Power,” World Nuclear Association. Accessed October 30, 2015. Available at: http://www.world-nuclear.org/info/Current-and-Future-Generation/Nuclear-Fusion-Power.
European Union, “Research boost for future fusion reactor,” Horizon 2020, July 28, 2015. Accessed October 30, 2015. Available at: http://ec.europa.eu/programmes/horizon2020/en/news/research-boost-future-fusion-reactor.
Raffi Khatchadourian, “A Star in a Bottle,” The New Yorker, March 3, 2014. Accessed October 30, 2015. Available at: http://www.newyorker.com/magazine/2014/03/03/a-star-in-a-bottle.
Raffi Khatchadourian, “A Star in a Bottle,” The New Yorker, March 3, 2014. Accessed October 30, 2015. Available at: http://www.newyorker.com/magazine/2014/03/03/a-star-in-a-bottle.
Subscribe
Get updates whenever new content is added. We'll never share your email with anyone.